Spotlight on Research: Transformational Research Through Modeling and Simulation
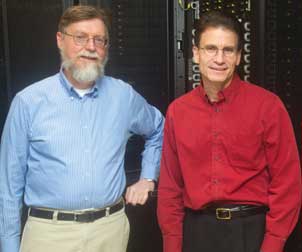
Pitt researchers tackle some of the most complex issues of our times in the University’s new Center for Simulation and Modeling.
Infectious disease. Global warming. The world economy. These are vastly different subjects, yet all have some things in common. All are complex systems influenced by multiple external stimuli. All have intense importance for humanity, deciding who lives, where they live, and how they live. And our understanding of all three can be advanced through computer simulation.
Researchers at the University of Pittsburgh are building powerful models to simulate complex phenomena. Armed with these new models and aided by the help of more and increasingly powerful microprocessors, they are asking essential questions that would have been unthinkable to ask computationally as recently as 10 years ago. Pandemics, climate change, banking meltdowns—all can be described by algorithms, and all can be simulated with powerful computers.
Growing cohorts of researchers from a broad array of disciplines have used computer modeling to tackle some of the biggest challenges in their fields. Yet, despite the phenomenal increase in the power of computers, traditional simulation tools have been limited because they do not adequately address multiscale processes occurring across large space and time scales.
A Giant Step Forward in Simulation and Modeling
This limitation is being addressed on a large scale at the University of Pittsburgh through the Center for Simulation and Modeling (SAM), a multidisciplinary research center established in Fall 2008, that works with Pitt researchers to develop new multiscale approaches for simulation and modeling. The center also helps foster collaborations across disciplines in modeling and simulation.
“Multiscale modeling has the potential to revolutionize the way science is conducted; to foster transformational research; and to stimulate the advancement of new technologies that can have an unprecedented impact on materials, energy, medicine, and many other fields,” says George Klinzing, Pitt’s vice provost for research.
SAM is codirected by Kenneth D. Jordan, Distinguished Professor of Computational Chemistry, and J. Karl Johnson, W.K. Whiteford Professor and Interim Chair in the Department of Chemical and Petroleum Engineering. The center has available a computer cluster with 2,000 cores connected by a high-speed Infiniband network, which greatly speeds up demanding calculations that require simultaneous use of multiple CPUs (Central Processing Units). A unique feature of the center is its team of PhD-level consultants with expertise in various facets of computer modeling and simulation. The consultants work closely with research groups, assisting in improving the performance of their computer codes and in making the transition to parallel computing platforms.
SAM engages in a wide range of other activities on campus, e.g., developing and teaching new graduate-level courses on scientific computing, organizing workshops on emergent computing technologies and on software applications of interest to researchers in multiple disciplines, and running a multidisciplinary seminar series on high-performance computing. An exciting new development is a partnership with the University’s Computing Services and Systems Development (CSSD) group in making available a new computer cluster with the latest-generation Intel and AMD CPUs as well as NVIDIA GPUs (Graphical Processing Units, which are specialized coprocessors that enable extremely fast floating point computations).
The center serves as a focal point where almost 50 faculty members (plus more than 100 graduate students) from diverse fields share their experiences and expertise with one another. The thematic focus areas of SAM are energy and sustainability, nanoscience and materials engineering, medicine and biology, public health, and economics and other social sciences.
Leaving the Serial World
Computers traditionally have operated serially, processing one line of code, then another, and another. But microchip speed is topping out, and chip designers are now putting more CPUs, or cores, on a single chip—meaning that even desktop computers have parallel computing capabilities. The benefits to this kind of programming are obvious: A simulation that takes a year to complete on one processor would take less than a day if one could get it to run efficiently on 512 processors.
“Leaving the serial world behind is no small task,” says Johnson, who adds that he grew up in a serial world. “The problem with the shift toward parallel programming is that very few people know how to do it, and there’s a huge demand for people who really understand it.”
When the University asked its faculty what they needed in a computational center, Klinzing says, their answer was, “We need high-level consultants; we don’t just need hardware.” In response to that need, SAM was created to provide in-house, PhD-level computational consultants who advise faculty and their students.
The center allows researchers from across the University to be able to tap more easily into experts like Liz Marai, assistant professor of computer science. Marai’s specialty is data modeling and visualization, the conversion of mountains of numbers into visual representations that can then be used for interpretation.
For example, Marai has recently teamed up with SAM as part of an interdisciplinary research group led by Caterina Rosano in the Department of Epidemiology in Pitt’s Graduate School of Public Health (GSPH).The project aims to provide practical computational tools for analyzing the correlation of mobility characteristics in older adults with brain pathophysiological processes. Marai and her students are developing advanced visualization strategies and tools for analysis of MRI data and their correlation to mobility measurements.
“We take numbers and measurements and try to generate insight,” says Marai. “We run a tight loop between data, visualization, and analysis.” Marai believes that the center has enabled computational research at Pitt to take a big step forward. “It fosters collaboration between experts from different fields. It’s not just adding computing power, it’s combining methodologies across disciplines to look at these really important questions—how galaxies are formed, how the human body works. Expert collaboration across disciplines can solve really big problems.”
Toward Energy and Sustainability
Other important questions include those being asked about energy, sustainability, and the environment.
Jordan uses models to analyze the structure of methane hydrate, a compound formed from water and methane under high pressures at low temperatures. It is most abundant in deep ocean water and under the permafrost near the Arctic. The methane embedded in these deposits contains more potential energy than all known oil and natural gas reserves on the planet. It also represents a major potential contributor to global climate change. Methane is a potent greenhouse gas, 20 times more potent than carbon dioxide (CO2), and, as the polar region warms, the melting permafrost may release the vast supply of methane beneath it.
Either way, understanding methane hydrate’s mysterious properties is crucial. Jordan is working with the U.S. Department of Energy’s National Energy Technology Laboratory to simulate the structure and dynamics of methane hydrate. In hydrates, water molecules form polyhedral cages around methane molecules. Simulations allow Jordan’s team to explore questions about methane hydrate that would be nearly impossible otherwise. “On the computer, we can study the network of cages without the methane molecules—something that cannot be done experimentally,” he says.
Jordan’s group also is studying a hydrate that is formed from water and CO2 molecules, which may be useful in sequestering CO2, the world’s most abundant greenhouse gas.
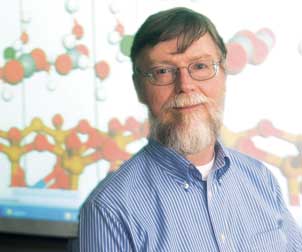
Like many researchers at the University, Jordan collaborates with experimentalists at numerous institutions, including Yale and Purdue universities, to combine his research with state-of-the-art experimental approaches.
Carbon dioxide also is an area of inquiry for Johnson, who is using computational modeling to understand how CO2 interacts with many other materials. Johnson and his collaborators are hoping that the materials they help to design will one day be used to capture CO2 and slow global climate change.
“For the next 20 to 50 years, we’re going to be relying heavily on fossil fuels for energy,” Johnson says. “How can we use that resource without releasing CO2 into the atmosphere?” Johnson’s group is modeling a class of nanoporous material compounds with “pores” 1/1000th the width of a human hair in diameter to understand how they interact with CO2. He hopes their modeling will lead to the design of a material that can capture CO2 from power-plant emissions. The captured CO2 could then be stored through carbon sequestration.
Modeling is helping one Pitt researcher to tackle another aspect of the energy challenge: how to make solar power more accessible. Geoffrey Hutchison, assistant professor of chemistry, uses computation to study a class of conductive plastics called polythiophenes. These materials can be used as photovoltaics and could become a cheaper alternative to silicon-based solar cells. Just as intriguing to Hutchison is that polythiophenes can be processed like inks. “You could paint this on the roof of every car and every building and have solar cells in all these places,” Hutchison says.
Hutchison is experimenting with these plastics on the computer, simulating conductive properties of different molecules. “We can look at what happens if we change, say, a carbon atom into a nitrogen—you can do that on a computer, and to do that experimentally would take months.”
Hutchison and his collaborators put together a group of 100 potential molecules and, through simulations, gleaned five or six that could be good candidates for solar cells. “A lot of these molecules look like things we could make, but we want to understand whether to put in the effort to make them,” he says.
Laura Schaefer, a professor of mechanical engineering and materials science, Bicentennial Board of Visitors Faculty Fellow, and deputy director of the Mascaro Center for Sustainable Innovation at Pitt, uses computer modeling to develop green alternatives to chlorofluorocarbons (CFCs) and related polymers.
CFCs have long been used as refrigerants in air conditioning because their chemical profile allows them to draw away heat from the air around them efficiently and safely. But CFCs deplete the Earth’s ozone layer, which blocks harmful solar radiation, and these chemicals will be phased out completely this year.
Hydrochlorofluorocarbons (HCFCs), the chemicals used to replace CFCs, are more environmentally friendly but still have a negative impact on the ozone layer. Regulators also will begin phasing out the most common HCFCs this year.
Schaefer, who also has used computer modeling to study the use of acoustics for refrigeration, is simulating how different combinations of chemicals would react to find a safe, efficient alternative. Her work involves multiscale modeling that predicts how the system as a whole will perform.
The urgency to develop a safer, more energy-efficient refrigerant is great; air conditioning accounts for one-sixth of U.S. household electricity use. Schaefer’s work is leading to new understandings in the basic science of fluid dynamics, she says. “The theoretical insights have been really fascinating. We’re learning a lot on how complex fluids behave at a small level. This could have far-reaching impacts for researchers in other fields.”
Tracking Global Health
Computer modeling tools have become increasingly important to understanding and addressing global health problems. By using informatics and computational modeling and simulation, researchers and policymakers can understand more about current public health challenges and determine the best strategies to prevent disease and improve human health.
Modeling also can show how disease spreads through whole populations, as demonstrated by the work of Donald Burke, GSPH dean, UPMC-Jonas Salk Chair of Global Health, and associate vice chancellor for global health at Pitt. Burke is a pioneer in using computer modeling to understand the behavior of pandemic disease.
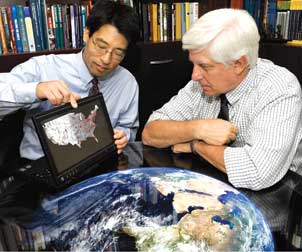
For decades, Burke tracked infectious diseases like HIV/AIDS and dengue fever throughout the developing world. In the 1990s, he began to think of pandemics as computable processes. “I now think of virus transmission as an algorithmic process, with underlying subprocesses and patterns,” Burke says. “I never would have thought that way before I started to use computer modeling and simulation.”
The computer model Burke and his collaborators designed to simulate an outbreak of avian flu in Southeast Asia—using transmission statistics from past epidemics, census data, and other social patterns—has helped the U.S. Department of Health and Human Services, U.S. Department of Homeland Security, and Centers for Disease Control and Prevention develop policies on travel restrictions, vaccinations, and school closures in the event of an outbreak. The Bill & Melinda Gates Foundation, which funds projects to help eradicate infectious disease, committed $10 million to Burke’s group to build a model for the use of vaccines to contain epidemic diseases.
“Computer modeling allows us to test in silicon our ideas on populations,” says Burke, and not just for epidemics of infectious diseases. Smoking, obesity, and drug use are all examples of public health problems where social behaviors spread from person to person in a fashion similar to contagious microbes and where modeling can help people think through and evaluate public policies designed to limit or reverse the spread of the behaviors, according to Burke. Pitt can be an international leader in this exciting new field, he says: “We’re definitely on the front edge. My intuition is that soon, virtually every aspect of public health research and policy development will be supported by modeling and simulation, and Pitt is extremely well positioned to lead the field.”
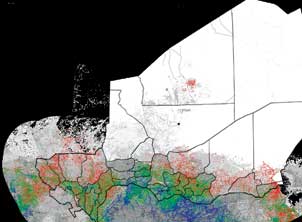
In 2009, the University was designated a MIDAS (Models of Infectious Disease Agent Study) National Center of Excellence, leading a collaborative network of scientists in the development and use of computational models that will prepare the nation to respond to outbreaks of infectious diseases. Recently, SAM consultants have teamed up with Bruce Lee, an assistant professor in GSPH and a core member of the recently founded Public Health Dynamics Laboratory. SAM is developing highly parallel computational modeling tools for studying patient and MRSA (a type of bacterial infection found commonly in hospitals) flow in human institutions such as hospitals and long-term care facilities.
Embracing Turbulence
Anyone who’s puzzled by an inaccurate weather forecast can appreciate why turbulence is one of the great problems of modern science. “Albert Einstein advised all his associates not to get involved with the problem of turbulence; it’s a very chaotic phenomenon,” says Peyman Givi, William Kepler Whiteford Professor in the Department of Mechanical Engineering and Materials Science and director of the Laboratory for Computational Transport Phenomena at Pitt.
Givi hasn’t stayed away from turbulence—he’s embraced it by developing unique methods of modeling the phenomena of turbulent combustion and high-speed combustion inside engines. He’s gained attention for a novel way to combine two modeling methods—one employing exact calculations and another using probability—to provide an accurate way for engineers to experiment with engines even before they are built.
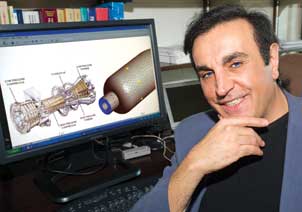
Rolls-Royce and NASA engineers already use Givi’s model to predict temperature differential, fuel usage, and emissions for different engine and fuel combinations. Givi’s models plot billions of fluid particles across hundreds of thousands of time intervals. It’s a lot of work, but it saves countless amounts of time and energy for engineers trying to create more efficient, cleaner-burning engines.
“When you design an engine, you’d like to be able to know how it will work before you build it,” Givi says. “Computer simulation allows people to do that.” Givi is working in collaboration with SAM consultants to develop a powerful computational software with advanced parallelization techniques to enable simulations of ever-larger systems with unprecedented fidelity.
Designer Materials and Nanotechnology
Just as Givi’s models depict how engines will work before they are built, computer modeling allows researchers to test the properties of new materials and novel chemical compounds prior to their being built.
“If you can understand what’s happening at the level of atoms, you can build things from the bottom up and create designer materials,” says Johnson. His lab is using models to probe the physical properties of carbon nanotubes—long, strawlike molecules with very narrow diameters that scientists think may be useful for separating different gases or liquids. For example, a carbon nanotube membrane that selectively separates water from sodium chloride could be used as an easy, low-energy way to desalinate seawater.
Johnson and his group model how specific mixtures of molecules interact with these structures, showing, for instance, how quickly these materials flow through the nanotubes. Unless the flow rate is fast, the membrane will not be useful in practical terms for separating fluids. The model created by Johnson and his collaborators found that gases would pass much more quickly through the nanotubes than expected. Laboratory results published in a 2006 Science article by a separate research team supported some of the predictions Johnson’s team made: The measured flow of gases and liquids was about 1,000 times faster through the nanotubes than through conventional porous membranes.
Jordan is using modeling to study one of the most abundant materials on Earth: water. “Although water is probably the most studied substance on Earth, we still don’t understand all of its properties, many of which are unique,” Jordan says.
Jordan’s group simulates the behavior of small clusters of water containing up to 100 molecules. These studies are shedding new light on a wide range of processes, among them chemical reactions in the atmosphere and electrochemistry. One of the most important problems on which Jordan’s group is working is whether charged particles, such as electrons and protons, prefer to be on the surface or in the interior of water clusters. This fundamental science question turns out to have far-reaching consequences, including those of environmental importance in atmospheric chemistry.
One researcher is using computers to model “sticky stuff”—wet or very fine granular material. Joseph McCarthy, a professor and William Kepler Whiteford Faculty Fellow in the Department of Chemical and Petroleum Engineering, studies the science of mixing sticky material. This is an important process in fields like pharmaceuticals, where a thimbleful of active chemicals must be mixed evenly into a roomful of flour-like substance. McCarthy and his collaborators build intricate models to see how best to mix these materials based on their size, density, and ability to be attracted to or repelled by water. They calculate the behavior and mechanics of billions of particles interacting over hundreds of thousands of time segments. This takes months to run across several high-powered processors. The time put into these models saves McCarthy and his colleagues from having to build time-consuming physical experiments.
The McCarthy group simulated a “chute flow” experiment, in which a substance is poured down a surface with a series of zigzags built into it. To construct a physical experiment, the team would have had to build a chute as high as 12-story Benedum Hall, which houses the Swanson School of Engineering. “We can make a lot of measurements you can’t do when you’re working experimentally,” McCarthy says.
Computer modeling is allowing Anna Balazs, Distinguished Professor of Chemical Engineering and Robert Von der Luft Professor, to explore the creation of nanomachines that behave in much the same way human cells do.
Balazs uses simulations to study how microcapsules—synthetic bubbles roughly the size of a human blood cell—moving across a surface could form an artificial “skin” on a damaged material. She also has simulated communication between these synthetic bubbles using a route similar to that used in processes occurring in cell signaling. These materials one day could form the basis for chemical sensors that “heal” defective surfaces.
“This artificial skin is essentially a coating that could indicate where a surface has been damaged,” Balazs says. She believes that her simulations will help experimentalists to follow the “recipe” for the nanomachines her team has created using computational modeling.
Unlocking the Body’s Mysteries
Modeling is becoming an invaluable tool for many Pitt researchers in medicine and biology. From studying proteins at the atomic level to focusing on the body’s immune system, models let these University researchers quickly test theories—enabling innovations that could help save many lives down the road.
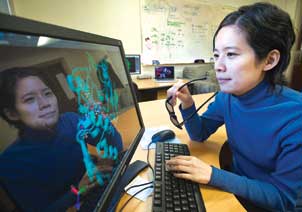
Lillian Chong, Pitt assistant professor of chemistry, uses computer simulations to study proteins, the body’s workhorses, in order to understand better the roles they play in the biological pathways of the cell. Chong studies natively unfolded proteins—so named for their seemingly disordered structure—which include a protein called tumor suppressor p53, thought to play a role in cancer. Proteins initiate important chemical functions in the body by “folding” into target molecules.
Chong’s group simulates the kinetics of these protein interactions on tiny time intervals of femtoseconds (one quadrillionth of a second). “There’s no way you could get that level of time detail without a computer,” Chong says. These simulations run through Folding@home, a unique collaboration based at Stanford University in which scientists use distributed computing to take advantage of idle computers around the world. They can run protein-folding simulations on a network of more than 600,000 idle personal computers and Sony PlayStation 3s.
The Center for Simulation and Modeling is helping researchers to share their expertise and experience with others at Pitt. “It makes a lot of sense to have a center that supports that kind of faculty collaboration so we can leverage that kind of expertise from so many people in this community,” she says.
Rob Coalson, Pitt professor of chemistry and physics, and his team model ion channels, one of the body’s least understood but most important protein structures. These nanoscopic channels enable the passage of charged atoms like hydrogen, potassium, and chlorine through the body and are essential for everything from the creation and propagation of nerve signals and muscle contraction to the production of such chemicals as insulin.
“Malfunction of ion channels is implicated as the root cause of many serious diseases and neuropsychiatric disorders, including cystic fibrosis, certain types of epilepsy, and migraine headaches,” Coalson says.
According to Coalson, it is difficult to test ion channels in their natural environment within a living organism, but it’s important that they be better understood, as about half of the drugs on the market today target ion channels and their protein cousins. Coalson’s group has developed simulations that calculate the rate of ion flow through channels in cell membranes for various transmembrane voltages and electrolyte concentrations in order to gain insight into the relationship between channel structure and function.
Through computational models, Ivet Bahar, John K. Vries Chair and professor of computational biology, and her colleagues in Pitt’s School of Medicine simulate the interactions of proteins with potential inhibitors, small compounds that can limit the undesirable activities of some proteins. In collaboration with the Drug Discovery Institute, Bahar’s lab members screen libraries of hundreds of thousands of chemical compounds for their potential to interact with target proteins in the search to identify promising drugs for further development.
By doing “virtual screening,” Bahar says, she and her collaborators can not only increase the likelihood of identifying more potent inhibitors but also speed up the process of drug discovery. Other members of the computational biology department also use computational tools to study the role of microRNA, tiny strands of genetic material, in regulating the immune response with respect to cancer and in understanding the complex biophysics of cell signaling.
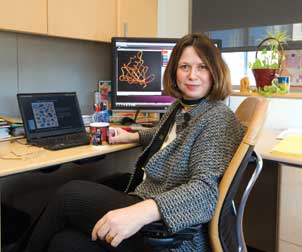
G. Bard Ermentrout, University Professor of Computational Biology and Pitt professor of mathematics, uses computational modeling to simulate complex medical phenomena. Ermentrout, who’s used modeling to study everything from the trailmaking properties of ants to the pigmentation design of mollusk shells, frequently collaborates with colleagues in the fields of medicine, neuroscience, and biology to study how certain patterns occur.
Ermentrout and his collaborators use models to understand the immune response during sepsis, a potentially fatal condition in which the body’s response to infection inflicts “collateral damage” on internal organs like the lungs. They are trying to model how the immune system response in sepsis reaches a tipping point after which the body’s own immune defenses start causing more harm than good. “At what point does the immune feedback become overwhelming and start to avalanche and cause problems?” Ermentrout asks.
Ermentrout also has used modeling to study phosphenes, the geometric visual patterns that occur when one looks at strobe lights, hallucinates, or enters a pre-epileptic state. He thinks that the patterns represent neural activity transposed directly onto the brain during those instances when the brain’s visual apparatus is knocked off its usual setting.
“There are some problems in science that really lend themselves to modeling,” says Ermentrout. “We’re trying to find the algorithms that generate these patterns.”
Forecasting the Uncertain
Predicting how the world economy will react to a laundry list of economic contingencies—wars, resource shortages, or banking meltdowns, to name just a few—is no task for the faint of heart. “The devil is in the details,” explains David N. DeJong, a professor and former chair of the Department of Economics who was recently named Vice Provost for Academic Planning and Resources Management. DeJong is trying to harness the power of computational methods to create economic forecasting models designed in part to chart reactions to such contingencies.
DeJong uses computer simulations to reconcile two branches of the field that don’t always communicate with one another: theory and statistics. Statistics are invaluable in unearthing the details of economic trends, but, DeJong explains, “They don’t really answer the question, ‘How come?’ ”
DeJong has already begun this task, mapping theoretical models of economic behavior onto statistical models. He understands that because models are simplifications of reality, there are some things that models will have difficulty accounting for: “No matter how rich our understanding of the aggregate economy is, we’re never going to get everything.” For instance, how does a researcher account for the reaction of consumers to unforeseeable changes in their environment not built explicitly into the model, like the recent foreclosure crisis or instabilities in the banking sector?
But as our understanding of the relationship between tax policy, investment, and consumption increases, DeJong and other economists increasingly will use structural models to read the economic tea leaves.
“What I’d like to do is to be able to answer questions like ‘Should the Federal Reserve Board be more worried about interest rates or inflation?’ or ‘What are the effects of a tax holiday going to be?’ I’ll fire up the model and give you an answer,” DeJong says.
Traditional Methods, New Tools
Researchers at Pitt will continue to refine their understanding of the complicated systems they’re modeling. They will collaborate heavily with experimentalists to test their theories against real-world data and explore new ways to make computational modeling even faster. In these ways, computational modeling is not so far removed from the most rudimentary rules of scientific inquiry that have been around for centuries: observe, experiment, and analyze.
As Givi says, “In almost anything we do in science, we use what we know and model what we don’t. The issue is how do you combine these two worlds to come up with a unified methodology? That’s where we are putting our efforts.”
Other Stories From This Issue
On the Freedom Road
Follow a group of Pitt students on the Returning to the Roots of Civil Rights bus tour, a nine-day, 2,300-mile journey crisscrossing five states.
Day 1: The Awakening
Day 2: Deep Impressions
Day 3: Music, Montgomery, and More
Day 4: Looking Back, Looking Forward
Day 5: Learning to Remember
Day 6: The Mountaintop
Day 7: Slavery and Beyond
Day 8: Lessons to Bring Home
Day 9: Final Lessons